Quantum computing is one of the most powerful tools developed in the past few decades. Quantum computers (QCs) solve otherwise unsolvable problems in physics and mathematics, all because of the unique properties of their design and construction. To understand why a QC works so effectively we’ll dive briefly into what quantum computing is, why cryogenics are essential in quantum computing design, and what qubits are and how they are used. Additionally, we’ll discuss a critical design piece that doesn’t get talked about as much: the importance of interconnect components in quantum computing designs.
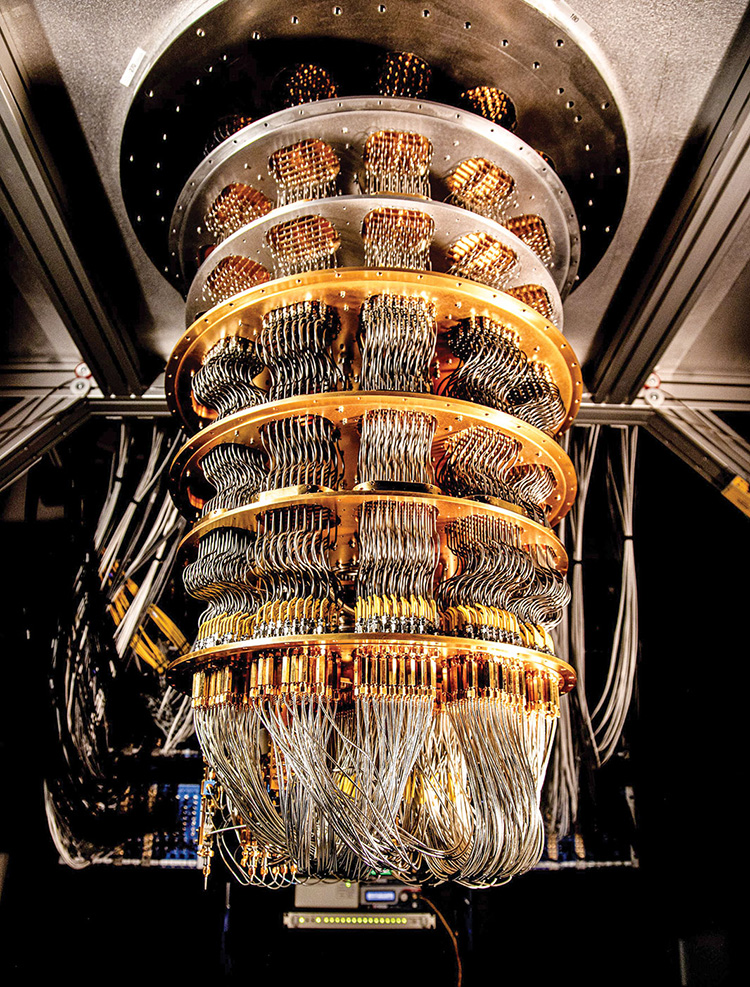
If you work in RF and Microwave you are probably familiar with the technologies surrounding Quantum Computing and the bourgeoning industry it has created. There is no shortage of articles, white papers, and product highlights from industry publications. Additionally, there are countless manufacturer announcements of products and technologies that support the insanely low temperatures of these mysterious marvels. Lastly, there is steady news media calling attention to the dangers QCs present to national security and data security should they be employed by “bad actors”. Yet, how much do the majority of us know about Quantum Computers? How much do we really need to know?
As it turns out, an advanced degree in physics is no more necessary to understand the components and functions of QCs than a degree in computer science is to know how a Classical Computer (CC) functions. We all use PCs/laptops, mobile phones, and video gaming units without deeper knowledge about how they function. QCs are strange and complex, but so are the CPUs we use every day with their billions of transistors networked inside of their architecture.
Many QCs currently operating are made of sub-systems that use the same components used in RF and Microwave systems that RFMW knows well. There are a few different approaches to how a QC functions, but what they all require are signal processing and interconnects, of which we have deep knowledge and experience to provide exactly what is required.
What is Quantum Computing?
Before going too deep, it is worth mentioning that QCs will never replace CCs. As you will read below, they are mind-bogglingly fast, but really only good at certain types of calculations. QCs are also far too complex for the tasks for which CCs are already exceptional. There is also the slight problem that physicists don’t truly understand how they work, but more on that later.
All CCs are based in the binary language of 1s and 0s, or more simply voltage on and off. When a program runs a function with a set of data, the calculations are executed one at a time. Even if multiple processor threads (parallel inputs & outputs) are used, they run one at a time.
In contrast, QCs can execute calculations for all input values at the same time. How is this even possible, you may ask? Quantum bits, or simply qubits.
Sub-atomic particles like electrons and photons are what are used as qubits. Electrons, for example, have a constant, random spin. They can be controlled with lasers or microwaves to force them to spin in two main directions: up and down (like the 1 and 0 of binary). Where this gets mind-blowing is that when the electrons are between up and down, they are essentially in both states simultaneously. Like the paradox of Schrödinger’s cat, a qubit can be observed as up and down, a phenomenon called Superposition. This is like adding a third bit to binary, which allows for more complex calculations.
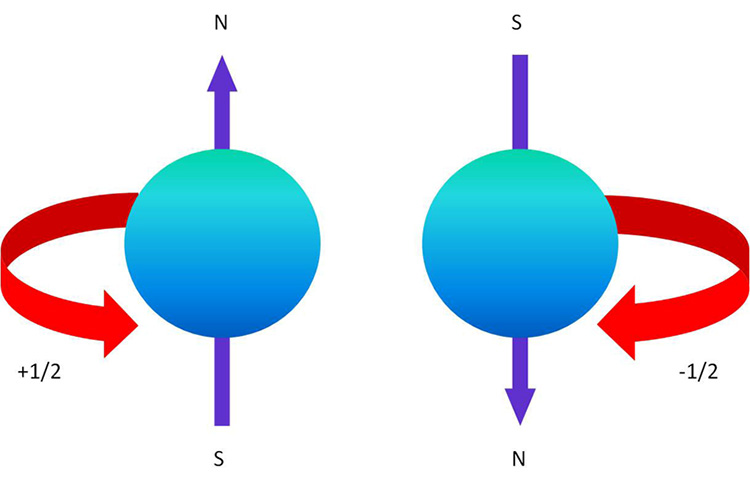
To make it more interesting, electrons like to interact with each other. If one electron changes spin direction, all the other electrons that it is “entangled” with instantly change at the same time: a phenomenon known as quantum entanglement.
When there are at least two electrons that can be up or down, there are at least four possible outcomes: up-up, up-down, down-up, and down-down. But, because of superposition, these electrons can be up, down, or both. When you throw in the “both” state of superposition, we can see that with every electron added to the entanglement chain, the possible outcomes increase exponentially. Specifically, 2n, where n is the number of qubits. Therefore, two qubits are 22 or 4 possible outcomes, four qubits are 24 or 16 possibilities, sixteen qubits are 216 or 65,536 possible outcomes, and so forth.
All of this science results in a processor with astonishing capabilities; a QC processor with 53 qubits was claimed to have completed a task in 200 seconds that would have taken the most state-of-the-art supercomputer 10,000 years to finish.
Why Are Cryogenics Used?
Now that we know how qubits behave, the challenge is controlling something so random and energetic as an electron. The answer is to take away as much energy from the qubits as possible and isolate them from any disturbances, or “noise” (e.g., external radiation, vibration, and other particles). This is where cryogenics—operation within the sub-120 kelvin range—comes into play.
Using the Google QC “chandelier” as an example (see Figure 1), the quantum processor and its interconnects are contained inside a dilution refrigerator (DR). This DR reduces the temperature in stages and protects the system from “noise” like vibrations and cosmic radiation.
Each of the disc flanges contain numerous interconnects and are hermetically sealed to separate adjacent stages. The temperature decreases at each stage down to the quantum processor (QP) experimental level, which measures a balmy 20-40 millikelvins (.02-.04 K). To put that into perspective, the coldest regions of outer space are 2.7 kelvin (-270°C, -455°F). By reducing the temperature that much, the qubits can be isolated and held in place long enough to shoot them with microwaves. However, the amount of time they remain isolated is incredibly short.
Our problem is we do not truly understand subatomic physics. Our current methods of controlling qubits are limited to as little as 20-40 nanoseconds and as much as 2-3 seconds before their entanglement devolves into decoherence. Once decoherence sets in, the qubit entanglement stops, and the qubits in the quantum processor can no longer be used. A new, extremely expensive quantum processor is needed. How is that for an expendable commodity?
How Are Qubits Used?
Just as a CC has a CPU, a QC has a Quantum Processor (QP) wherein the algorithms are executed with input data and output the results. However, the design and function of the QPU is quite unique. The Superconducting QC processor utilizes transmon qubits captured in quantum buses, connected to each other and the PCB by resonator lines. The resonator lines are designed to transport analog waveform signals in and out of the processor.
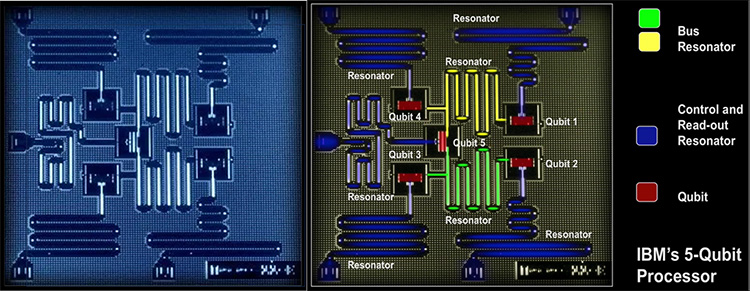
At the PCB, those signals are routed to and from the QP, through board-level signal conditioning components, and to dozens of coaxial interconnects. The coaxial cables and connectors run through every stage of the DR to pass the analog signals from the control and compute equipment to the QP and back.
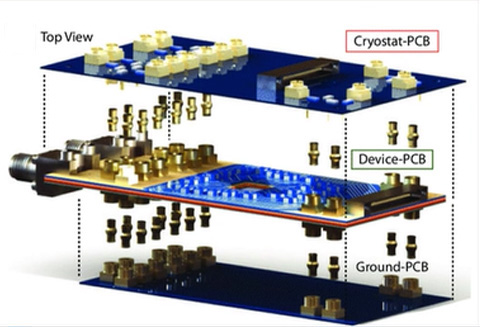
Importance of Interconnect in Quantum Computing
There are many challenges with designing Quantum computers, and today we’ll focus on one that doesn’t get talked about enough: intelligence in your interconnect.
Each section of the computer must transmit signals to the next through an increasingly harsh environment of the DR until you reach the QP. It’s important that the different layers of essential interconnect components separate the progressive cooling stages of the DR and do not degrade the signal performance in either direction. Otherwise, the analog data coming from the qubits, which are already highly error prone, is plagued with errors from noise, reflectance, crosstalk, and attenuation.
For example, let us assume that you have the perfect quantum processor functioning with unbelievable precision in your cryogenic chamber. However, some of your cables, connectors, amplifiers, adapters, etc. are not specifically designed for the temperatures in that environment. This could lead to material failures that degrade the signals with reflectance and phase delays or interfere with the qubits themselves with magnetic field from connector alloys.
How important is interconnect when designing quantum computers?
First, let’s take a look at a diagram of the five main function “layers” of a practical Quantum computer, courtesy of IEEE Spectrum:
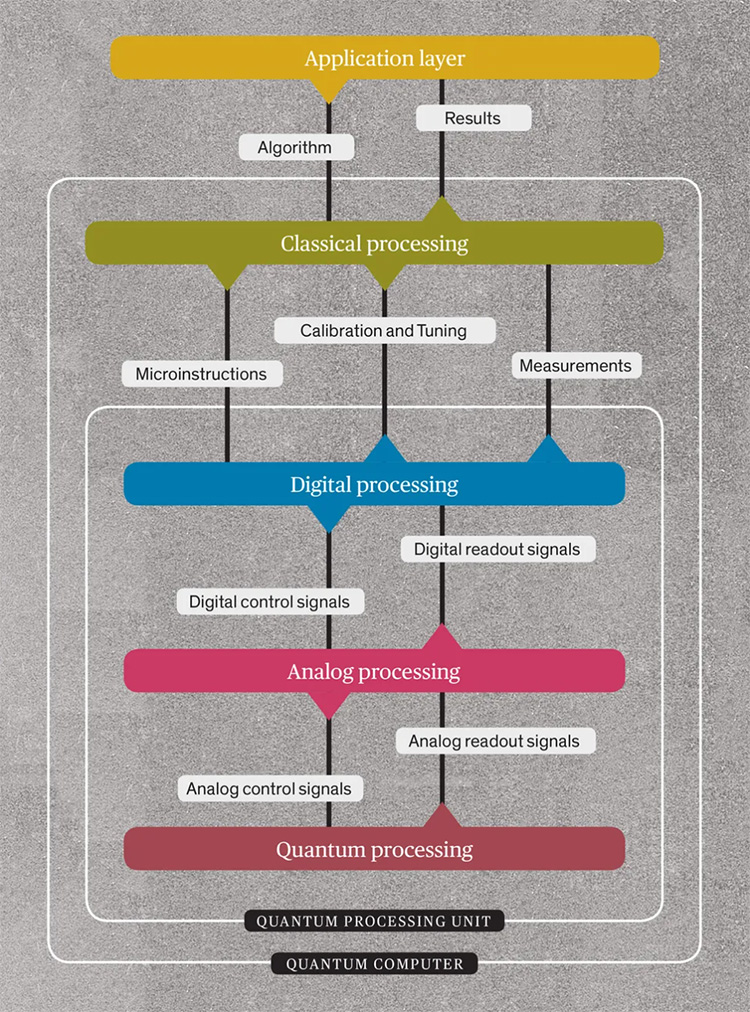
We made our own version of Chad Hagen’s drawing to show where RF and microwave components play a critical part in the design.
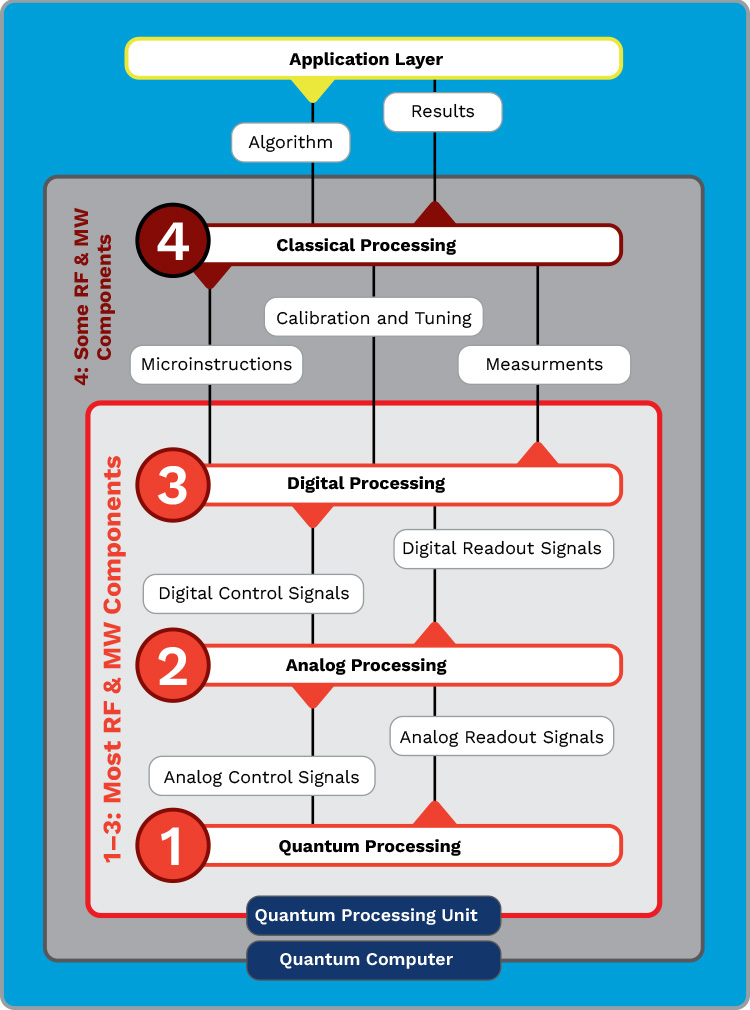
Interconnect Components Within the RF and Microwave Heavy Quantum Computer Layer Cake
- Layer 1 Quantum Processing Interconnect Components: Flexible Coaxial Cable Assemblies, Superconducting Semi-Rigid Coaxial Cable Assemblies, Non-Magnetic and Superconducting Coaxial Cable & PCB Connectors and Adapters, Cryogenic Attenuators, Cryogenic Terminations, Cryogenic Isolators & Circulators, Cryogenic Amplifiers, Cryogenic RF Coaxial Switches, Cryogenic Bias-Tees
- Layer 2 Analog Processing Interconnect Components: Flexible Coaxial Cable Assemblies, RF & Microwave Adapters & Connectors (Bulkhead + Panel), Low Noise Amplifiers, Attenuators, Terminations, Filters, RF Coaxial Switches, Bias-Tees; Couplers
- Layer 3 Digital Processing Interconnect Components: Flexible Coaxial Cable Assemblies, RF & Microwave Adapters & Connectors (Bulkhead + Panel + Board-to-Board), Filters
From an RF and Microwave perspective, we are most interested in the portion of the QC dubbed the Quantum Processing Unit (QPU), which incorporates three layers: Quantum Processing (1), Analog Processing (2), and Digital Processing (3). There may be some RF/MW components in the Classical Processing (4) layer, but certainly not to the level of the other three.
Layer 1: Quantum Processing
In the first layer (1), the QP and signal routing PCB reside in the coldest part of the DR. All of the PCB connectors, filters, bias tees, and cable assemblies must be non-magnetic to avoid interfering with the qubits with an induced magnetic field caused by the microwave signals. They also need to withstand the temperatures of the DR. At cryogenic temperatures, all materials become brittle and can fail from fracturing, but some metal alloys can go through a change in the arrangement of their molecules and expand. The tight machining tolerances of small metal connectors can actually expand as a result and cause cracking in mated connectors or the PCBs they are attached to.
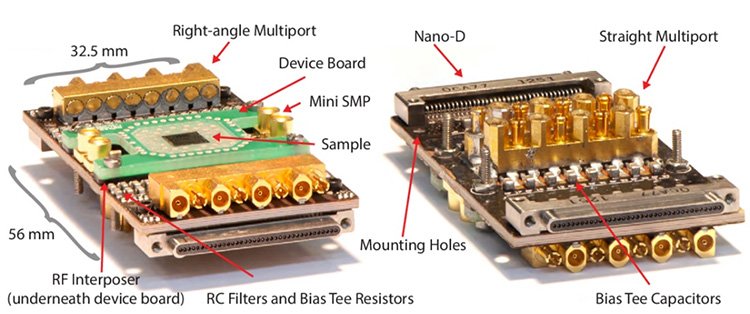
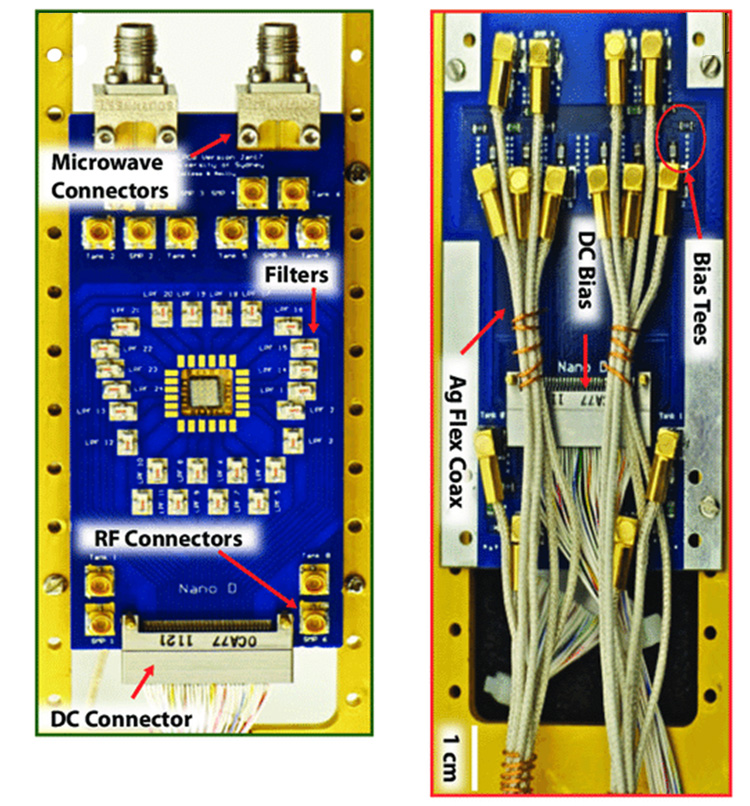
Reputable companies like Rosenberger produce high quality connectors, multi-port connectors and adaptors manufactured with superconducting alloys like Niobium-Titanium and non-magnetic semi-rigid cable assemblies that are shielded with stainless steel and Cupro-Nickel to ensure there are no failures or interferences.
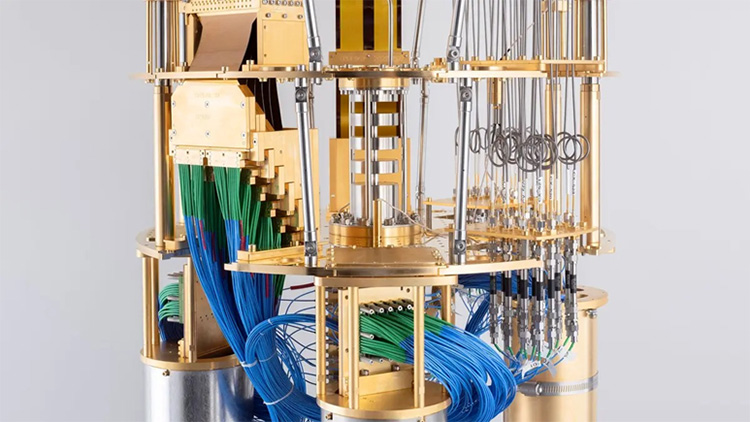
Throughout the chambers of the DR there are machined flanges with hermetically sealed glass feed throughs that separate the temperature reduction stages. Within those stages, there may be attenuators and terminations needed to test and adjust the signal data. Companies like XMA are focusing on attenuators and terminations produced specifically for cryogenics and Quantum Computing.
Within the DR, engineers may find themselves needing to isolate and test individual signal channels or utilize redundant lines in the event of a failure. In this instance, Radiall has developed a line of cryogenic RF switches. Based on the unmatched performance of their Ramses switches, these cryo-specific switches are designed for millions of switches with no degradation of the switch actuators and produce a maximum magnetic field of 0.75 mT.
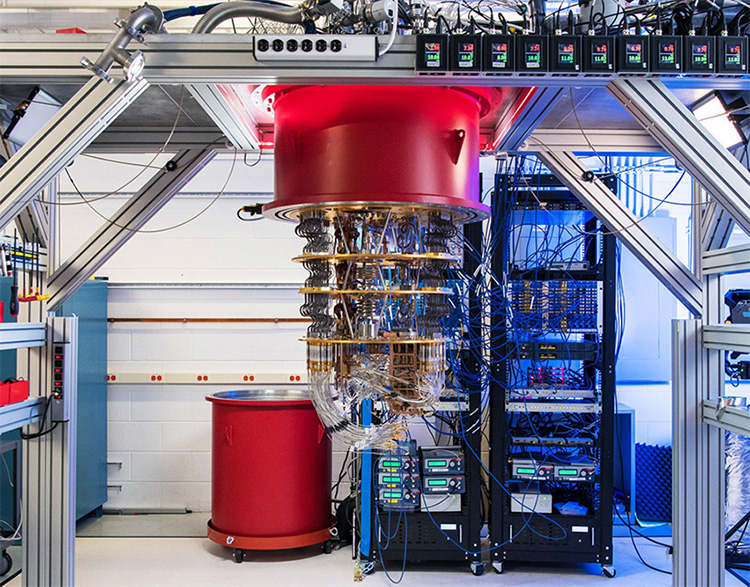
Layer 2: Analog Processing
Moving out of the DR, analog processing is the second layer (2). The qubit controller instruments here send control and data signals to the QPU through the numerous coaxial cables feeding into the DR and receive the analog readout signals carrying the computed data from the qubits. The data waveforms received from the qubits are raw and require filtering, shaping and error correction, which may require numerous quantum analyzers. All of these controllers and analyzers rely on high quality coaxial cable assemblies to route the signals with low attenuation and high phase stability such as the TestPro from Radiall or APSK from Rosenberger.
Layer 3: Digital Processing
Continuing to the third layer (3), the analog signals and data need to be converted from or into a digital signal. The analog control signals start their life as a set of software instructions from the application layer in an algorithm, then converted to instructions from the classical processing layer. The digital processor converts the digital instructions to analog signals that the qubits can “understand”. Again, there are numerous coaxial cable assemblies carrying these signals to and from the analog processing units that must be high-quality, and low noise to ensure the quality of the signals.
In addition to the coaxial cables used, all the companies manufacturing the control, analysis, and conversion hardware require PCB and panel connectors, cable assemblies, switches, and more. If we look at each layer in Figures 9 and 10, the variety of interconnect products required is intense. There are many places where an inferior, incorrect, or poor-quality interconnect product could fail or introduce errors to an already error-prone technology.
Solution: Intelligent Interconnect
This might go without saying, but keeping interconnect components in mind through each stage of the design process goes a long way in building a reliable Quantum Computer. Here are a few key pieces of advice for teams approaching a new design:
- Select appropriate interconnect components that are designed specifically for each of the different layers and components of the quantum computer, as opposed to adding them as an afterthought. There is nothing worse than finding out the interconnect that was sourced at the end does not fit, has too much attenuation, or leaks signal into the rest of the system
- Talk to a technical sales expert. The assumption is that they are either not necessary or just trying to make a sale. It would seem that all of the information we need to make informed decisions is available online and once the right part has been selected, it can be bought online. The problem there is that the true performance of an interconnect may not be discovered until it is used, many weeks after it finally arrived after a long lead time. A trusted technical sales engineer will know far more about their products and the products of their competitors. They are concerned with winning your long-term business by building a trusting relationship as the subject matter expert who can get everything you want and nothing you don’t need.
- If you have component questions, ask a value-add distribution partner like RFMW to help you get answers. They can help source the appropriate products for your design from top-tier suppliers, ensuring ease-of-design and production success. If they do not carry it or cannot source what you need, they can leverage their decades of knowledge and relationships to have it developed and custom built.
Summary
Hopefully you have learned a bit more about how Quantum Computers function, why cryogenics are used, and why harnessing the properties of qubits is one of the most astounding breakthroughs in modern physics and engineering. You also know more about the design challenges that this technology presents, and why selecting interconnect products with the best materials and performance is so crucial to withstanding the rigors of such sensitive and demanding application.
RFMW carries and sources the non-magnetic and cryogenic-focused interconnect products designed specifically for Quantum Computing. We have the knowledge and experience to help you find out what you need to make your design succeed in this bourgeoning industry. Please contact Kevin McCormack: kevin.mccormack (at) rfmw (dot) com or Dan Smoliga: dan.smoliga (at) rfmw (dot) com.
More Articles from RFMW about Quantum Computing
